Paul Dirac named these particles “bosons” to honor Satyendra Nath Bose, an Indian scientist who developed the idea with Albert Einstein. The discovery of the Higgs boson on July 4, 2012, marked a turning point in physics. With it, the fundamental component of the cosmos that gives everything its mass has been identified. But 10 years later, there are still many unresolved concerns regarding this special particle called the “god particle” and its impact on the foundations of our conception of the physical universe.
Our planet would likely not exist at all if it weren’t for the Higgs field and the Higgs boson that goes along with it. Several physicists, including Robert Brout, Francois Englert, and Peter Higgs, proposed this as early as 1964. The expected particle has now been discovered as of 2012. However, the Standard Model of particle physics still has a few significant holes. Additionally, the Higgs boson has in some ways generated more questions than it has resolved. So, what’s the next step?
What Makes the Higgs Boson Special?
The absence of mass would result in a cosmos devoid of atoms and other forms of ordinary matter. The fundamental constituents of matter stick together and interact with one another only because of the mass of the particles that make them up. But from whence does the mass of the constituent particles come? For a very long time, the Standard Model of particle physics—the foundation of our conception of the physical universe—did not provide a solution.
The Weak Nuclear Force
Additionally, there is a difficulty with the bosons, which serve as the basic forces’ carriers. According to theory, they should not have any mass, unlike fermions that create matter, such as quarks and electrons. Photons and gluons are also examples of carrier particles of electromagnetic and strong nuclear forces. They have no mass. Photons may thus travel at the speed of light without encountering any obstacles.
The weak nuclear force, however, does not work with the plan: On the one hand, it contains two carrier particles, the W and Z bosons, instead of one. And these bosons, on the other hand, have a mass. This explains the weak nuclear force’s limited range and how it works in radioactive decay. However, for many years, it was unknown how and why only these exchange particles, but not the others, acquire mass.
The Scalar Field
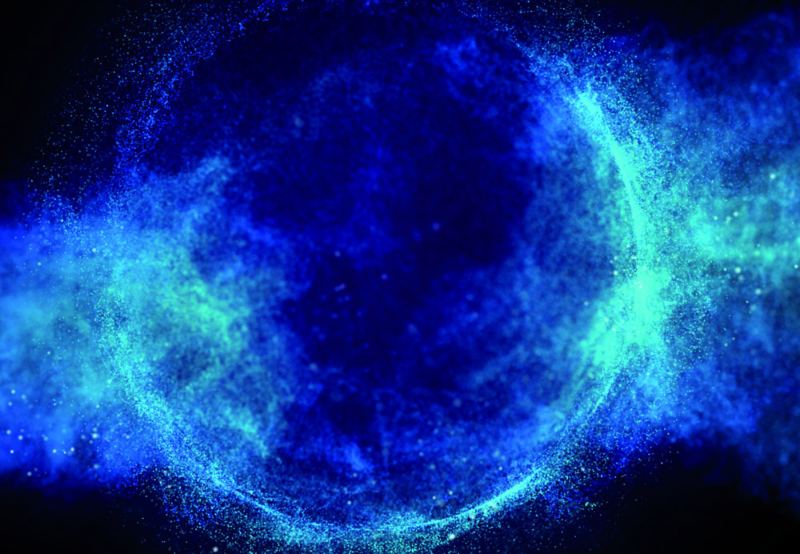
Further light was shed on the issue only in the early 1960s, when numerous theoretical physicists, including Peter Higgs in Great Britain and Robert Brout and Francois Englert in Belgium, started looking for a solution. They independently concluded that the issue may be resolved by an unseen field that permeates the whole cosmos. Some of the constituent particles may interact with this scalar field in quantum interactions, modifying their characteristics. So, they can only move by using energy, and because of this, they have mass.
The Brout-Englert-Higgs mechanism is often compared to a cocktail party by the British physicist David Miller. A throng of other guests swiftly congregates around a significant celebrity as soon as it enters the room. Due to the crowd, the celebrity is unable to travel very far—much like a particle with a large mass that can only be propelled with a lot of energy.
Asymmetrical Effect
The Brout-Englert-Higgs mechanism also explains the fact that not all carrier particles have mass: The Higgs field has an asymmetry; it does not interact with all bosons uniformly. The carrier particles of these forces, photons, and gluons, remain massless since they are neutral regard to electrodynamics and quantum chromodynamics. However, the field has a kind of braking effect on all other particles and gives them mass.
This is comparable to how fur has its hair growing in one direction: a particle, like a photon, feels no resistance and maintains its masslessness if it flows along the Higgs field’s “hairline.” On the other hand, when a particle goes against the flow, more energy is needed, and the particle accumulates mass. This holds for all fermions that may form matter, as well as for the W and Z bosons of the weak nuclear force.
The Higgs Field
That makes up the theory. But how can you back it up? The Higgs boson enters the picture here. Because if such a scalar field exists, it is capable of condensing at certain locations. The Higgs field may also appear as a particle at these locations, just as a photon is both a wave and a particle. “What else can be keeping the agreement between the Standard Model and the data just as good as it is? If there is not a Higgs boson, the theory does not make sense at all.” Peter Higgs said in 2004.
Even then, the physicist was sure that this missing component of the Standard Model’s puzzle would soon be discovered. The Higgs boson was sought after.
How Was the Higgs Boson Discovered?
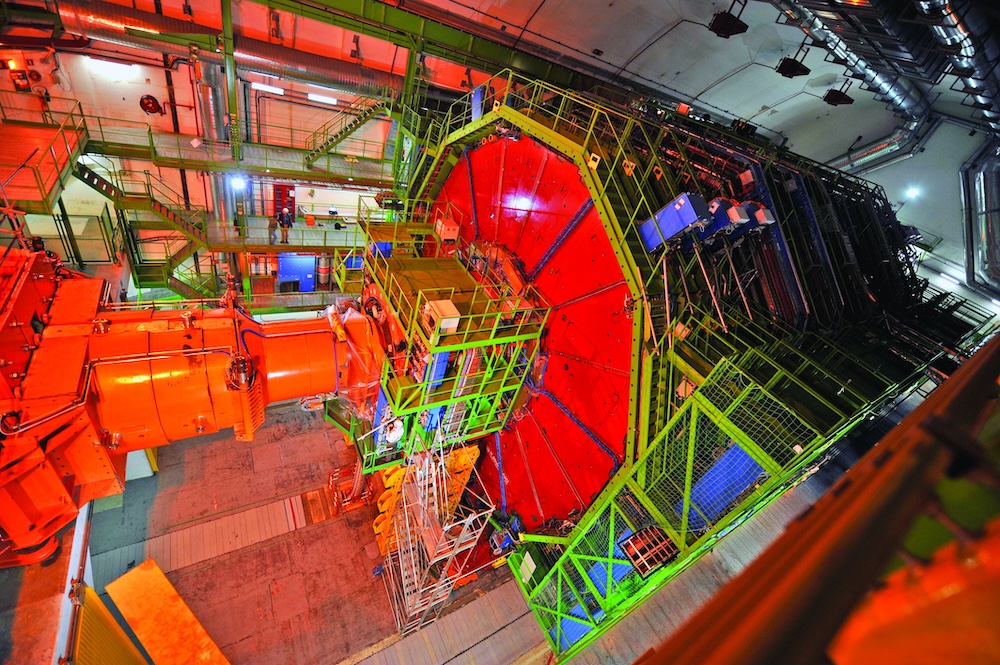
The Higgs boson was ultimately discovered in 2012 after almost 50 years of worldwide investigation by scientists. But why was it so challenging to find this missing Standard Model particle?
The issue was that the theory did not predict the characteristic that was essential for the experimental finding of the Higgs boson. These theories predict that this particle should just have mass and not spin or charge. But it was unknown how big this mass was. Only 18 megaelectronvolts to 800 gigaelectronvolts were within the range that could be used. The energy required to accelerate a particle is indicated in electron volts. This also applies to an elementary particle’s mass.
This has the following implications for the search for a particle: If you know the mass, you also know the energies at which you must fire particles at each other to generate the particle you are seeking in a collision. But when it came to the Higgs boson, researchers were stumbling about in the dark, and, on top of that, particle accelerators could only produce collision energy up to a certain point. Since these tests had previously shown that the Higgs boson would likely need to be heavier than 114 gigaelectronvolts, this was not entirely surprising.
Locating a Needle in a Haystack
The quest for the Higgs boson received a significant boost in 2008 with the start of operation of the Large Hadron Collider (LHC) at the CERN research facility close to Geneva. Because the finest circumstances for eventually discovering the sought-after particle were provided by this biggest particle accelerator in the world’s high-energy proton collisions. If the universe took the shape of the Higgs mechanism predicted by the Standard Model, there was no way to hide once the LHC got going.
The Higgs boson, however, decays back into the matter in less than a trillionth of a second and is only produced in around one out of a billion proton collisions. As a result, it cannot be directly viewed or quantified. Only the decay products it leaves behind may be used to identify it. Sadly, they are made of elementary particles like pairs of photons, muons, or Z-bosons, which are also released when the other collision products break down.
Therefore, finding the Higgs boson’s signature among all of these millions of particles is more like seeking out a specific haystack than it is like hunting for the fabled needle. The seemingly impossible procedure was made feasible by carefully analyzing which decay products, based on known mechanisms, the detectors should find, and then examining if there are deviations from this anticipated curve in a mass and energy range. If the Higgs boson was involved, there would be a little extra of the things that happen when it breaks down.
Unevenness in the Curve
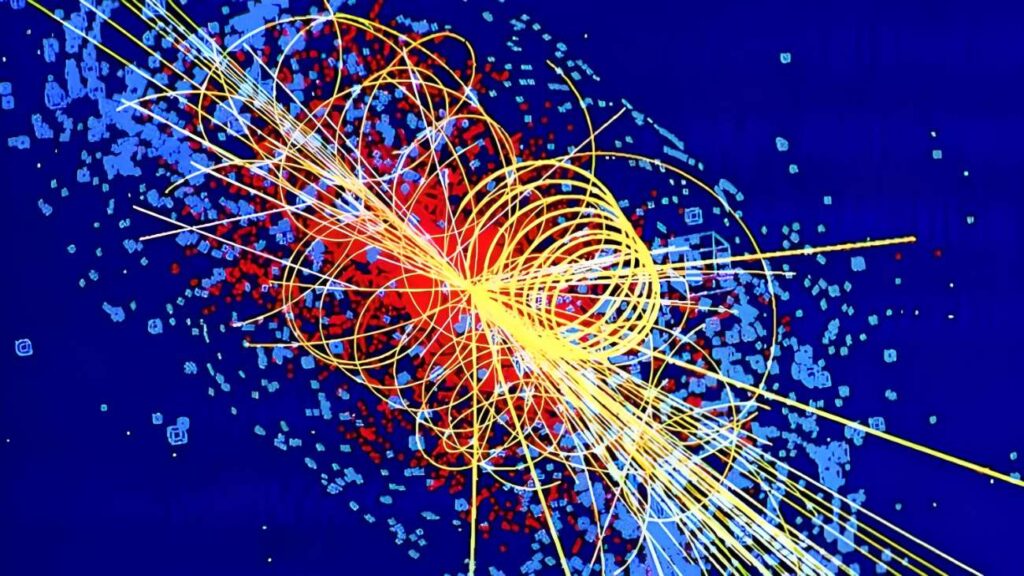
The same thing was seen at the LHC, at not only one but two of the big detectors: On July 4, 2012, representatives from the ATLAS and CMS consortia made their long-awaited announcement in front of the world’s media: the distinct signature of the Higgs boson has been found independently at both detectors. This could be found by looking for a “hump” in the decay product curve that is caused by the photon pairs or Z bosons that are made when the Higgs decays.
More than five standard deviations of significance were attained for both outcomes, which equates to a likelihood of nearly 3.5 million to one that the results are not just coincidental. The data from ATLAS and CMS satisfied the criteria for the official discovery of a particle with this value. Their findings indicated that this particle likewise required a mass of around 125 gigaelectronvolts. This matched the exact mass range in which the Higgs boson was thought to be present based on past searches.
Breakthrough in Physics
The Higgs boson, the last piece of the Standard Model’s jigsaw, was discovered after decades of research. Its discovery proved both the existence of a cosmic scalar field that provides mass and the Brout-Englert-Higgs mechanism, which was proposed more than 50 years ago.
For scientists throughout the globe, the discovery of the Higgs boson constituted a significant turning point. Peter Higgs and Francois Englert were awarded the 2013 Nobel Prize in Physics for their work. The hundreds of experimental physicists at CERN who participated in the search, and the theorists who had already passed away, were left empty-handed since the Nobel Prize may only be shared by a maximum of three live individuals.
The Particles Next to the Higgs Boson
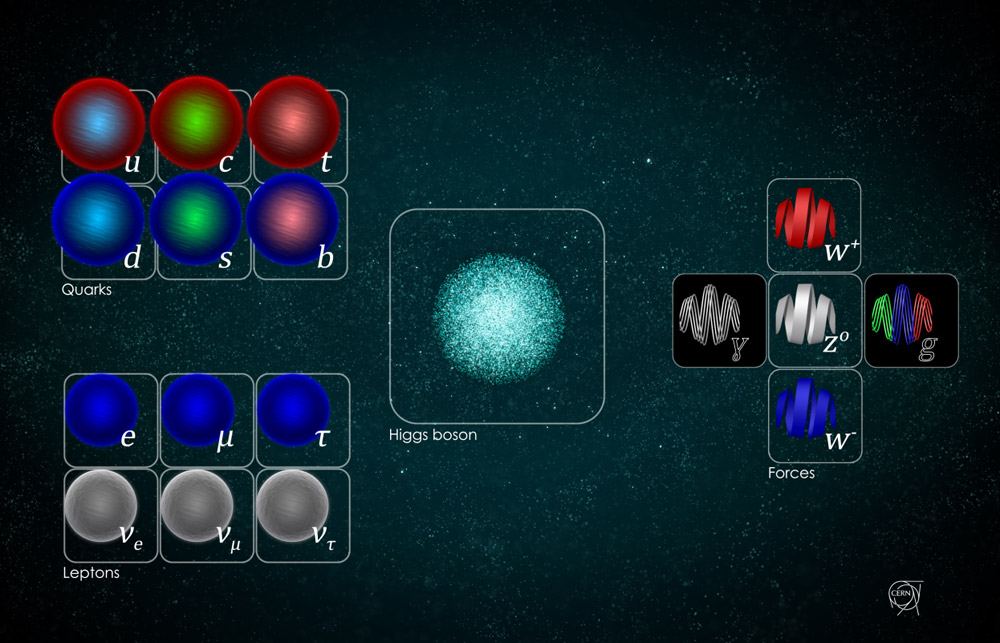
The Higgs boson is the cornerstone of the Standard Model, so everything that is discovered about this particle is important to the basic rules of physics. One of the most important questions about the Higgs boson’s interactions after its discovery was: Does the Higgs couple with other particles in the manner predicted by theory? Its discovery involves interactions with photons, W bosons, and Z bosons in each of the decays that were investigated. But the Higgs would also need to interact specifically with quarks and leptons if it is the “mass-giver” particle we are seeking. The latter include the heavier “siblings” of the electron, the muon and tau lepton.
The Higgs boson’s decay into bottom quarks and tau leptons allowed researchers at the LHC to discover the first of these interactions in 2016. This demonstrated that the Higgs truly interacts with matter particles as well as the fundamental force’s carrier particles. It took a little longer to find the Higgs boson’s most frequent decay: this preferred decay of the Higgs into two bottom quarks should occur 58 percent of the time.
But amid the “haystack” of many particles created by proton collisions in the particle accelerator, it was impossible to identify this decay phase. The evidence was finally available in 2018, when the ATLAS detector and the CMS detector at the LHC both produced data that demonstrated the Higgs boson’s decay into two bottom quarks with a significance of more than five sigmas.
A Strong Competition
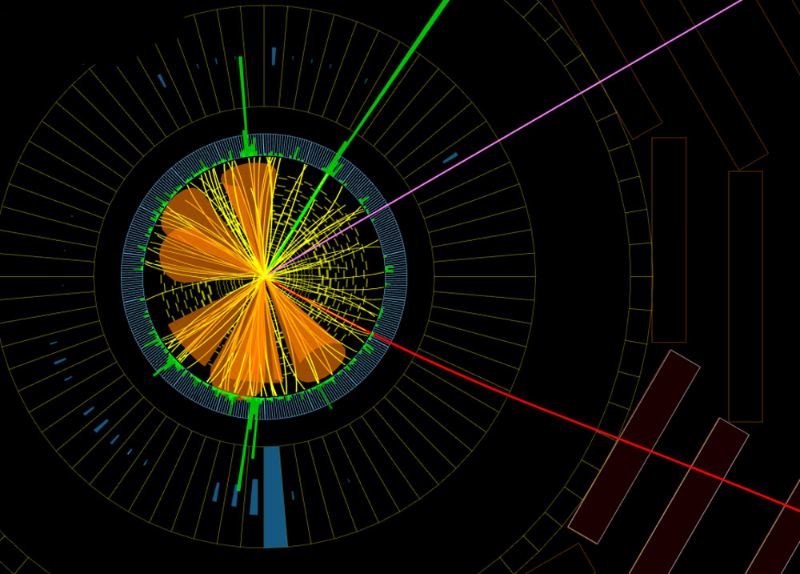
The Higgs boson should have the greatest coupling to the top quark, the particle that makes up the bulk of matter, according to the theory; only then does it get its huge mass. If true, certain proton collisions at the LHC should result in the production of a Higgs boson, together with a top quark and an anti-top quark.
However, this so-called ttH production method is exceedingly uncommon; just 1 percent of the Higgs bosons are formed together with top quarks. The only way to identify top quarks is via the decay products they leave behind since they are not stable. As a result, it took until 2018 for the measurements at the LHC to be of sufficient relevance. This was the first time that this mechanism had been empirically proven. The scientists had now witnessed all of the Higgs boson’s interactions with third-generation heavy quarks and leptons, as well as all of the significant ways that the particle was produced.
From the “Higgs Portal” to Dark Matter
However, interactions between undiscovered elementary particles and those specified by the theory might be much more intriguing: The Higgs boson, unlike other particles in the Standard Model, has no charge nor spin and may therefore interact with neutral particles, including types of bosons that have not yet been identified. Many scientists believe that such a neutral particle might be the long-sought dark matter particle.
We also refer to this potential as the Higgs portal. This makes the Higgs a terrific instrument for the quest for dark matter. Some Higgs decay products appear to be missing from some bosons in the LHC could be one way to find these “dark” interactions. When neutral “dark bosons” that cannot be identified by detectors are produced during the process, they either escape unnoticed or cause an imbalance in the known decay patterns.
Physicists at CERN may have discovered some early proof of recognizable divergences thus far. But they have a long way to go before they can find them or pinpoint exactly what they are. During the third run of the LHC, which has started, they are hoping for better data and higher Higgs production.
The Higgs Field’s Hidden Properties
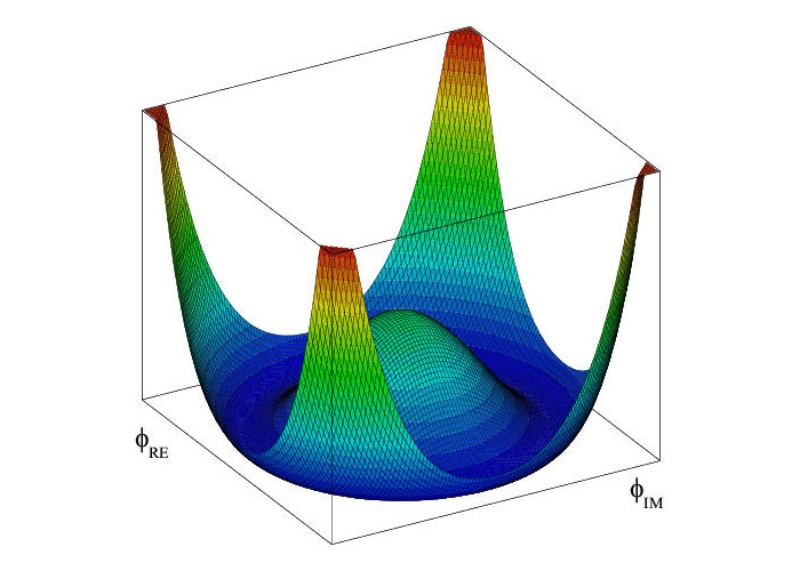
CC-by-SA 3.0)
The Standard Model of Physics now has a substantial hole that the Higgs boson has filled. But even though it has been found, there are still many questions about it, not the least of which is what the Higgs field and its particles are made of. The Higgs boson is often presented as the particle that completes the Standard Model but in practice, it brings up a ton of new issues.
Spontaneous Symmetry
One of them addresses the history and characteristics of the Higgs field. The Higgs boson is the first and only scalar particle among the basic forces of existence. The Higgs field, in contrast to other forces, has no preferred direction and is consistent even when reflected. The theory states that this field was produced immediately after the Big Bang, long before atoms were ever thought of.
Initially, the Higgs field’s scalar values averaged zero everywhere. Nothing and no one had mass as a consequence. The Higgs field, however, experienced a spontaneous symmetry breach only a few fractions of a second after the Big Bang, adopting a new configuration whose values, on average, amount to an energy of around 246 gigaelectronvolts. It started to affect other fields and particles, giving some of them mass. The counterintuitive aspect of this is that although the physical world is not symmetrical, the equations defining this field are nonetheless symmetrical.
Physicists also use the sombrero (the Mexican hat) or champagne bottle models to explain this spontaneous symmetry breakdown of the Higgs field: The scalar field looks like the curving sombrero or champagne bottle’s upturned bottom. The field’s design allows for complete symmetry, such that everything would balance on the middle elevation if a pencil were placed there. However, in practice, the pencil topples over relatively rapidly and falls in one direction, breaking the symmetry—at least for the pencil—even while the field’s form remains unchanged.
Beyond the Hat’s Brim
The issue with this scenario is that it is not yet apparent if or how the Higgs field can be accurately characterized by the Sombrero model and how it continues once past the brim of the hat. Is it possible that there is a chasm beyond it where the field might take on even lower values than in the valley of the “hat brim”? The field would only be metastable at that point and may shift into a brand-new, lower energy state. The Higgs field would be stable if the values of the “hat brim” kept expanding outward.
The so-called triple gauge boson couplings—a characteristic that represents the interaction of the Higgs boson with its own peers—is one method of providing an answer to this query. According to the theory, the Higgs particle may be the only one in the Standard Model to interact with itself. In the particle accelerator, some Higgs bosons would have to decay into two more Higgs bosons if this were the case.
The frequency and energy at which this triple-coupling happens, if it does exist, will be crucial in determining whether the Higgs field behaves as predicted by the Standard Model or if there is an opportunity for “new physics” in the form of undiscovered particles or forces. Scientists aim to have more and better data in the third run of the LHC, which started on July 5th, 2022. The ATLAS team at the LHC has already started hunting for the decay products of such triple boson production.
Divisibility of the Higgs
And the ATLAS collaboration’s researchers are presently looking into yet another query about the Higgs boson: Does the Higgs boson even have a substructure or is it a true, indivisible fundamental particle like photons, quarks, and gluons? This is predicted by several theories of physical processes beyond the Standard Model. These models suggest that the Higgs could more closely resemble the pion, a particle made up of an up quark and an anti-down quark that mediates the bonding between protons and neutrons in the atomic nucleus.
The production of unusual “vector-like” quarks would be a sign of such a substructure of the Higgs boson. The first analyses of the collision data from the LHC were presented by physicists with the ATLAS collaboration in 2021. But as of now, these findings show that the Higgs boson operates as predicted by the Standard Model, proving that it is a real elementary particle.
But the study of the Higgs boson and its quirks has only just started. Because if we can detect the properties of the Higgs, then it will address some of the hottest concerns in physics.
Future for the Lhc and the Higgs Boson
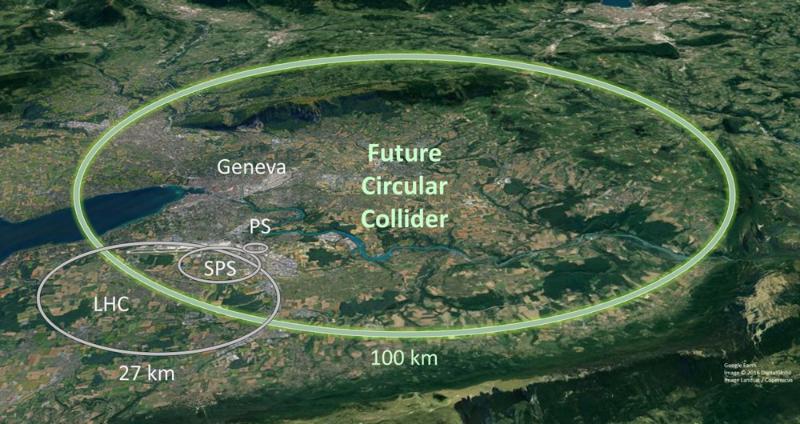
The Higgs boson’s discovery was a significant achievement in physics. However, significant advances in particle physics have not been made since, and many important topics remain unresolved. It has taken a while for physicists to finally see the abundance of new particles and scientific advances they had hoped for, particularly from the second run of the LHC and the discovery of the Higgs boson. For now, the LHC has mostly discovered the Higgs boson and the Higgs field it carries.
But in reality, there are still a lot of issues that physics has to address. Because there are still significant gaps in the Standard Model, even with some ambiguities clarified by the Higgs boson. For instance, Albert Einstein attempted to provide a true explanation of how the fourth basic force, gravity, relates to the other fundamental forces, but he was unsuccessful. It is also unknown whether gravity contains a carrier particle similar to those found in the other fundamental forces.
It is also unclear why, immediately after the Big Bang, the universe did not collapse once again. Because at that time, equal and oppositely huge amounts of matter and antimatter should have been created, and they would have destroyed one another. Yet, it is clear that the great annihilation did not occur since we would not be here now. Because of this, scientists believe that there must have been a small distinction between the properties or behaviors of particles and their antimatter counterparts. But thus far, searching for it has been fruitless.
Dark Energy and Dark Matter
Additionally, dark energy and dark matter—two components whose existence is still entirely unknown—constitute about 95 percent of our universe. The expansion of our universe is being driven by dark energy. Astronomers and physicists are still unsure of how it does this and why cosmic expansion is speeding up.
Dark matter is almost as enigmatic but a bit more researched. According to what we know about it, it must exist practically everywhere in the universe, including our solar system and the halo of the Milky Way. The velocity and form of galaxy clusters and galaxies are influenced by their existence. However, dark matter’s nature is likewise unknown since gravity is essentially its only mode of interaction with regular matter. It is still unclear what kinds of particles dark matter is made of and if a boson like the Higgs may be responsible.
Some basic issues concerning behavior and characteristics in the field of particle physics remain unanswered. One is the supersymmetry theory, which states that every known particle should have an additional, heavier, unnamed partner. However, there has been no sign of these hypothetical particles in the data as of yet. The proponents of this theory had anticipated a glut of such supersymmetry particles even from the initial runtimes of the LHC.
Scientists are still debating whether neutrinos exist in a fourth, sterile form in the case of these “ghost particles.” There are still some unresolved contradictions in the electroweak interaction, which results from the coupling of the electromagnetic and weak basic forces. People look to the Higgs boson and how it decays for more information, especially in this area.
All of these occurrences could be caused by “new physics”—processes and particles that have not yet been discovered. However, this has so far been well disguised from the physics community’s view. Certain obvious discrepancies have been found by physicists at the LHC and other particle accelerators, such as in the magnetic moment of the muon or B meson decays. The main issues, however, still have not been resolved.
The third run of the LHC at CERN is now expected to provide at least some answers. Because it is significantly more potent, numerous collisions could at least confirm and aid in elucidating the oddities previously picked up in hints. The maximum 13.4 teraelectronvolts that the LHC can produce in proton collisions is insufficient to explain many of the phenomena that are currently unsolved. More energy and alternative techniques are needed.
“Future Circular Collider” (FCC)
Due to this, some follow-up initiatives using more potent and substantial particle accelerators are already being discussed. The “Physics Beyond Colliders” initiative at CERN is investigating the future of accelerator rings. A 100-kilometer acceleration ring is also being developed for the years after 2040. Electrons and positrons will first collide and operate as a “Higgs boson factory” in this “Future Circular Collider” (FCC). The Higgs products are simpler to study since they smash with less energy yet create fewer interfering particles.
Later, the FCC will host proton collisions with energies of up to 100 teraelectronvolts. In the shape of an electron and positron ring accelerator of comparable scale to the CEPC, China is likewise preparing to build its version of the Higgs factory. A linear accelerator has been discussed in Japan, but it is unclear if the government will contribute to its funding. Some physicists are placing their hopes in novel kinds of mini particle accelerators that accelerate electrons with the use of plasma lasers in addition to the massive, pricey facilities. Their energies are often modest, but they are cheap, portable, and might be utilized to specifically examine certain sub-aspects.
It is too soon to say which of these initiatives will be carried out and which will succeed. But it is evident that to find new physics outside of the Standard Model, we must use every tool at our disposal, including astrophysical data, modest experiments at lower energies, and massive high-energy particle accelerators.
Bibliography
- Notes on Dirac’s lecture Developments in Atomic Theory, 1945
- The Bose in the Boson. The New York Times.
- Higgs boson: The poetry of subatomic particles. BBC News.