Changes in the weather, the conflict in Ukraine, and the gas shortage: Even more so in these uncertain times, a steady energy supply is necessary. Germany continues to insist on a nuclear phase-out, while other nations, like France, China, and the United States, want to increase their nuclear reliance in the near future. Small Modular Reactors (SMRs), which provide a small fraction of the energy of a huge nuclear power plant, are where their hopes are anchored. Can this technology really provide a solution, and how does it actually work?
Approximately 440 nuclear power stations are functioning around the world. They have an output of several thousand watts and need constant maintenance and upkeep. It is consequently not always safe to utilize such power plants, particularly in locations with poor infrastructure and an unpredictable power supply. Small Modular Reactors, a decentralized and, in theory, low-maintenance option from the 1950s, might actually be reintroduced today.
Initiated as a means of propelling nuclear submarines, the concept has now been adapted to address the growing energy crisis. How do the sodium and molten salt reactors function, though? What difficulties do operators have?
What Are SMRs and What Do They Promise?
Climate change is becoming worse, but the spread of renewable energy is also moving at a snail’s pace, for example in Germany. As a result, some argue that delaying the nuclear phase-out is a reasonable short-term solution to the problem of transitioning away from fossil fuels. The United States and France, among others, want to meet their climate goals in part by increasing their use of nuclear power.
Large Barriers to Entry
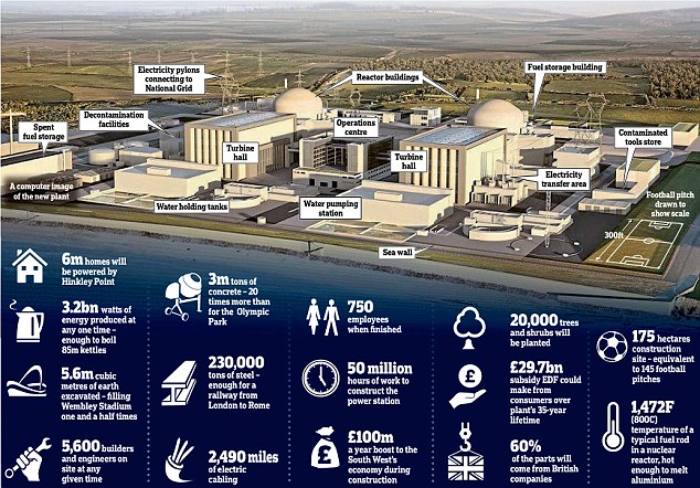
Presently, thirty nations generate nuclear electricity. More than two dozen more nations are considering adopting nuclear power, reports the International Atomic Energy Agency (IAEA). The fact that not every area meets the requirements is a major obstacle. This is because of the ongoing construction of very massive and complex nuclear reactors. They not only need a lot of room, but also reliable power and water supplies to function properly. To ensure the security of the power plants, a particular degree of experience in terms of staff is required.
Furthermore, the hefty initial investment expenditures act as an additional barrier. The cost of building the Flamanville nuclear power station in France, for instance, has ballooned to about €20 billion from an original estimate of €3 billion. The new estimate for the British Hinkley Point C nuclear power station project is around 27 billion euros, roughly six billion euros higher than the projection.
The Solution to All Problems
The advent of Small Modular Reactors (SMR) promises to solve all these issues. What we propose here is using a huge number of smaller nuclear power facilities rather than a few massive ones. These may then provide a smaller but more dependable portion of the electricity in a distributed fashion. The idea itself is not novel. Though the concept of using a distributed network of tiny reactors to secure the power supply dates back to the 1950s, it was never implemented at the time for technical reasons.
However, the idea seems to finally be coming to fruition after multiple failed efforts to bring it back. Nearly 70 types are in various stages of development right now. Despite the fact that no commercial SMR is currently operating, the IAEA reports that there are over 80 projects in the planning or development stages. Nonetheless, research reactors have already been deployed in China and other nations, and serial manufacturing of these reactors is expected to begin in 2030.
Low Output But High Volume
Typically, the output of such a tiny reactor is between 100 and 300 MW, although there are other versions that can generate 400 MW or as little as 30 MW. The biggest nuclear power facilities in the world can produce up to 3,500 megawatts, while on average they produce closer to 1,500 megawatts. Thus, about five to seven SMRs may serve as a stand-in for a single conventional nuclear power station.
Thousands more tiny units would need to be produced to replace the 440 or so nuclear power reactors already operating globally and to significantly increase the availability of nuclear electricity. The providers of this technology, however, claim that this won’t be an issue since the ideas are modular. Because of their manageable size, SMRs should lend themselves well to assembly-line manufacturing, on-site assembly, and eventual transfer to their ultimate destination. The cost-effectiveness of the product, in the long run, should also be assured via series manufacturing.
The primary emphasis in SMRs is on sodium-cooled and molten salt reactors, in addition to the already prevalent pressurized water reactors that are merely downscaled. How do these concepts work?
Molten Salt Reactors
The so-called Molten Salt Reactor (MSR) is a kind of nuclear power plant that is part of generation IV nuclear power plants, which are still in the research and development stage. Instead of using water, they employ a liquid salt solution.
This has the benefit of keeping the pressure within the reactor at a minimum, which improves security. When a pressurized or boiling water reactor fails, the water is released all at once, exposing everyone around to very high levels of radiation. In the Fukushima nuclear disaster of 2011, for instance, hydrogen explosions occurred from the hot steam and damaged many reactor buildings, spewing hazardous material into the environment.
The Benefits of Liquid Salts Are Many
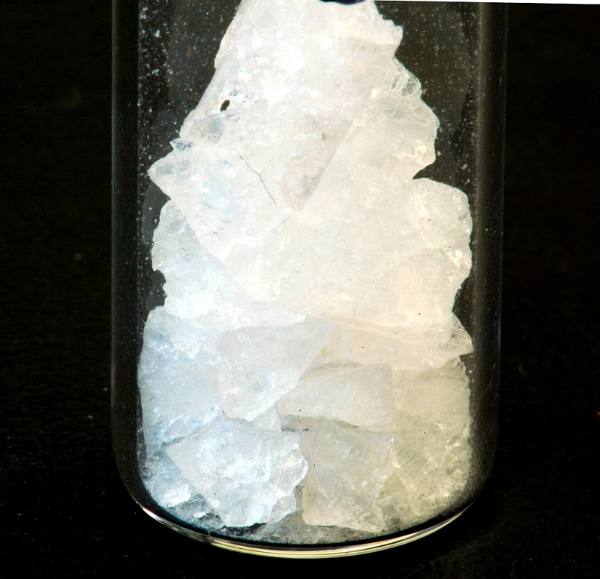
The molten salt reactor fuses the functions of fuel and coolant to avoid this. Uranium-235 is the primary fuel in nuclear reactors in the same way it is in traditional reactors. In addition, plutonium-239, a byproduct of the dismantling of nuclear bombs, may be utilized as a fuel source. The radioactive materials are not added to the coolant as oxides, like they are in fuel rods. Instead, they are added as salts of fluoride or chloride.
Equivalent parts of lithium fluoride (LiF) and beryllium fluoride (BeF2) salts (creates the molten salt FLiBe) are now the most promising coolant. Its 858 degrees Fahrenheit (459 degrees Celsius) melting temperature is lower than that of many other metals, which is a benefit. Because of this, there is less of a chance of the coolant crystallizing at junctions and causing a disruption in the circuit. The low vapor pressure of the material is another benefit, since it reduces the possibility of explosive expansion even at temperatures of around 1,832°F or 1000°C
Molten salts provide a significant challenge due to the tremendous corrosive impact they have on the surrounding materials. When it comes to this, FLiBe should be the deciding factor. Since beryllium reduces the molten salt’s redox potential, its corrosive properties are greatly diminished. The coolant’s transparency in its liquid condition is an added bonus that may be used to keep an eye on any contaminants.
The Structure of the Molten Salt Reactor
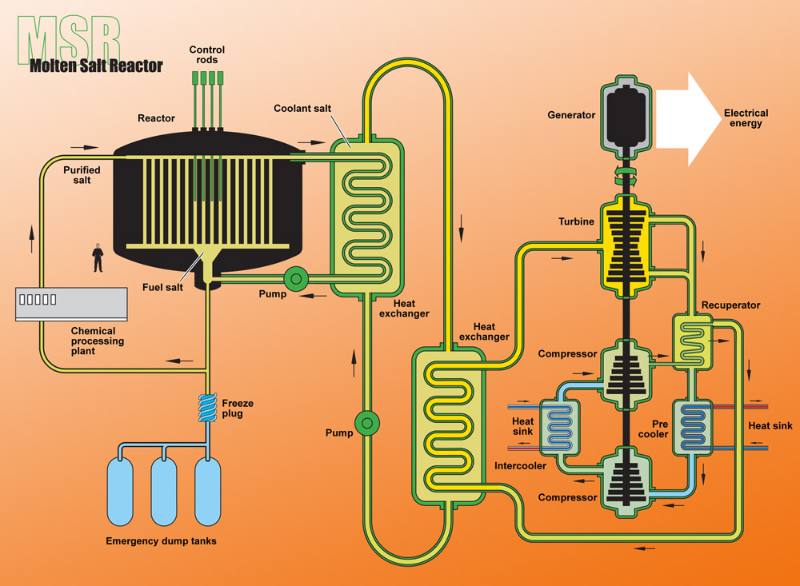
From the reactor chamber (top left), the liquid salt and fuel combination is piped to the first heat exchanger. By way of a third and final mechanism, the heat is carried through a second liquid salt circuit and transformed into electricity. The fuel is discharged if the passive safety valve (lower left) melts from overheating.
Numerous circuits make up a molten salt reactor’s basic structure. First, gasoline and coolant are combined and circulated through graphite rods that regulate the engine’s operation. As with traditional reactors, they are utilized as moderators and may be deployed with relative ease.
The reaction mixture’s heat is transferred to a second molten salt circuit through the first heat exchanger. This is contained inside the enclosure but does not contain any fuel. This is done so that any leaking heat exchangers won’t introduce any unwanted contaminants. Second, the heat is transferred to a steam generator to create power through a third cooling circuit.
Safety Through Passive Valve
Because of their exceptional safety, molten-salt reactors are often categorized as SMRs. In the event of an emergency, conventional pressurized water reactors need round-the-clock monitoring and the presence of qualified safety staff. In a molten salt reactor, the core is already liquid, so it can’t melt down, which is one of the worst things that can happen.
On the other hand, overheating is also a possibility. A water-cooled valve in the reaction circuit melts at unsafe temperatures, diverting the radioactive mixture to a series of cooling tanks. Each one of them is just big enough to stop a critical mass from forming. After the salts have cooled, they may be disposed of without risk.
Thorium-based Breeding
An additional use of a molten salt reactor is as an incubation reactor. The addition of thorium-232 to the fuel source accomplishes this. It undergoes beta decay from protactinium-233 to fissile uranium-233 upon neutron capture. Compared to uranium, thorium has the benefit of being more plentiful, and its disintegration creates fewer transuranics, leading to less long-lived nuclear waste.
There is a risk of uranium-233 contamination, which is a drawback of the breeder process, also known as the LFTR (Liquid Fluoride Thorium Reactor). It’s simpler to work with than weapons-grade plutonium, and it’s often regarded as the best nuclear bomb material. The LFTR is capable of producing reasonably pure uranium-233 thanks to the continuous separation of protactinium-233, which boosts reactor efficiency. The potential for this danger to be mitigated is enhanced by the fact that selective contamination of the fuel, such as with uranium-238, is possible.
Sodium-cooled Reactors
Fast Neutrons Ensure High Yield
Different uses are found for the water in both pressurized and boiling water reactors. As it circulates around the reactor core, it removes heat and acts as a coolant. More than that, it reduces the intensity of the neutrons produced by nuclear fission. Consequently, uranium-235 is able to absorb these hot neutrons. But this isotope forms just a fraction of less than one percent of naturally occurring uranium, therefore, enrichment is required before it can be used as fuel.
Fissioning uranium-238, the most prevalent isotope, requires so-called fast neutrons rather than thermal neutrons. This neutron is captured by a uranium-238 atom, which then decays via neptunium-239 to plutonium-239, the latter of which is used as fuel. During this process, more neutrons are emitted than are collected, resulting in an excess of fissile material. For this reason, versions of these reactors are referred to as “fast breeders.” Fast, however, has nothing to do with how quickly things are made but rather how quickly the neutrons travel.
Selecting an Appropriate Coolant
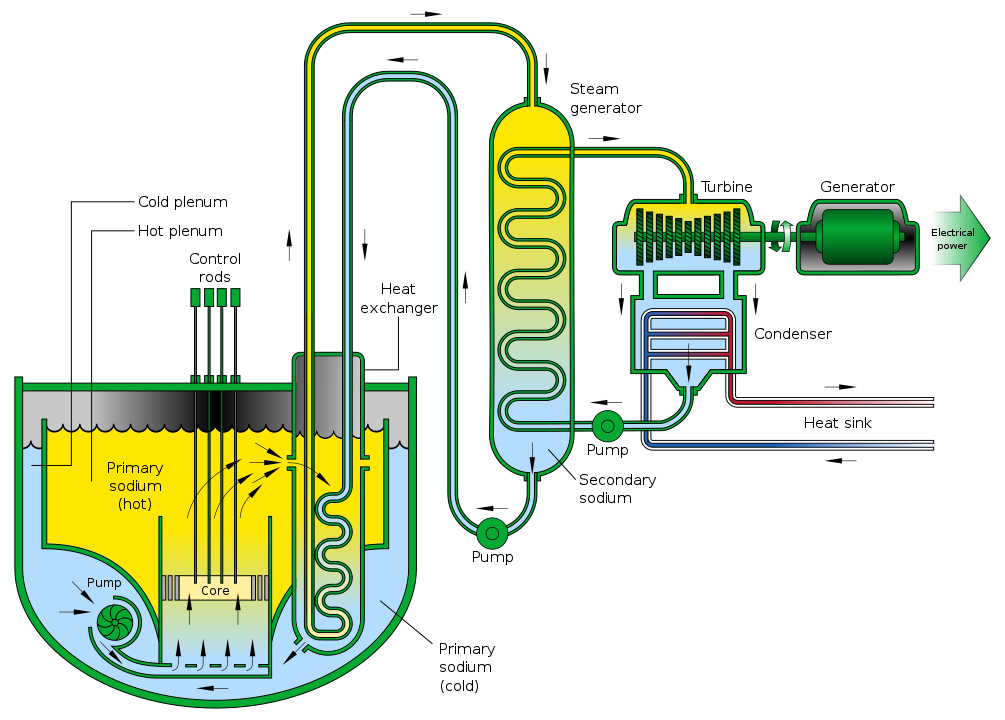
The sodium liquid is all around the reactor core (lower left). The first heat exchanger is situated in a sodium pool towards the center of the structure. A third circuit is used to create electricity.
Liquid sodium is utilized as a coolant in fast breeder reactors, which keeps the emitted neutrons from slowing down. It can be melted at 206°F (97°C) and heated to 1635°F (890°C) without changing its physical properties. Since it doesn’t need any additional pressure during usage (like molten salts), there’s no need to worry about explosions.
There are two distinct layouts for sodium-cooled reactors, known as pool and loop. The nuclear core of the reactor is immersed in a pool of liquid sodium in this configuration. A heat exchange system is also situated in the pool, and this fluid is circulated around both. The liquid sodium in the first heat exchanger is used to power the turbine in the third circuit, which in turn powers the electricity generator.
The only noticeable variation in operation between the standard design and the loop version is the location of the first heat exchanger. As opposed to a single pool serving as a boundary between the reactor room, the first circuit, and the heat exchanger, this design has dual circuits. A dedicated tank houses the heat/cool exchange mechanism.
A Dangerous Game
Because they utilize natural uranium, sodium-cooled breeders seem to be a viable alternative to light-water reactors at first glance. Not only does using many tons of sodium add up financially, but there are also significant safety concerns for those who work in power plants due to the usage of the alkali metal. Most of them stem from sodium’s extreme reactivity; when it comes into contact with oxygen or water, the alkali metal ignites violently.

Although the idea dates back to the 1950s, relatively few fast breeders have been run thus far due to the hazards involved and the high technological requirements. The French Phénix nuclear power station, which served the region around Avignon from 1973 until 2010, is a good case in point. It was the precursor of the 3,000-megawatt Superphénix, which went into service in 1986, but only had a thermal output of 563 MW.
Very effectively, the Superphénix sodium-cooled breeder showed the issues with using sodium as a coolant. A leak happened just a year after the plant was put into operation, resulting in the loss of 20 tons of sodium. After the second leak in 1990, all 400 tons of sodium had to be cleaned, a procedure that took eight months due to the fact that even trace amounts of contaminants may block oxides. Part of the roof caved in that year due to the weight of the snow, an argon leak occurred in 1994, and the power plant was eventually shut down in 1998.
The Emergence of a New Upswing
However, the idea of sodium-cooled fast reactors did not die with the Superphénix. Sodium pool power plants are being constructed or upgraded in a number of countries, including India, China, and Russia. SMR-based scaling up has the potential to produce breeder reactors that are both smaller and more amenable to precise regulation.
In general, fast breeders are seen as secure, with the exception of the dangers presented by sodium. The likelihood of neutron capture by the uranium also rises as the core overheats. This passively avoids a core meltdown by decreasing the reactor’s heat output.
However, much as with thorium reactors, breeder reactors raise safety issues due to the conversion of natural uranium to plutonium-239. The weapon-grade plutonium might be extracted and utilized in nuclear weapons.
Problems Regarding to the Small Reactors
Despite the novel technology and promises made by SMR proponents and the designers of the tiny nuclear power plants, several professional views and research point to potential hazards and difficulties with the reactors.
The most prominent is that the standards for preventing accidents are mostly derived from work done with water-cooled systems. For this reason, technologies like liquid salt or sodium-cooled reactors have not yet been well fitted to the so-called probabilistic safety assessments (PSA), in which the probability of specific occurrences is computed. Researchers say that a number of the factors that determine how reliable the systems are now will need to be recalculated.
Another issue is the vast quantity of relatively few reactors that would be required. While the variety of undesirable outcomes may be reduced in current SMR designs, the sheer number of possible locations still raises the probability of an accident. The danger of nuclear power plants grows in direct proportion to the quantity of electricity they generate.
Manufacturers Promise Too Much
Other SMR manufacturers’ worldviews are at odds with reality as well. Because of factors like the negligible quantity of radioactive material and passive safety ideas, only a small region is expected to be impacted in the case of an accident. However, certain researchers warn that in the case of an emergency, contamination might spread well beyond the plant’s physical location.
When it comes to water-cooled high-temperature reactors, institutions likewise disagree with the manufacturers’ assessment. The second group proposes coating the fuel with silicon carbide and a carbon shell rather than using elaborate confinement in their models. At temperatures below its failure limit of about 2900°F (1,600°C), radioactive isotopes may actually pass through this. As a result, there is no assurance that no radiation will be emitted in the case of an accident.
“Guarantees” Are Not Enough
It is also claimed that the self-regulating characteristics of fast breeder reactors and molten salt reactors are inadequate. It is true, for instance, that from a strictly physical point of view, in plants with fast neutrons, a rise in temperature provides a lower reactivity coefficient, which rules out core meltdown. However, scientists insist that a separate shutdown mechanism be mandated.
Consequently, there is also a liability question here: who is to blame if anything goes wrong in a power plant that is, according to the manufacturer, completely safe? For instance, a financial guarantee for the operators that would pay for the harm in this event is now under consideration. Experts agree that no safety concept, not even passive ones, can be considered a guarantee. For instance, the systems may get damaged due to outside factors, reducing their usefulness. This is why a secondary layer of protection is generally recommended by specialists.
Do Smaller Reactors Mean Less Waste?
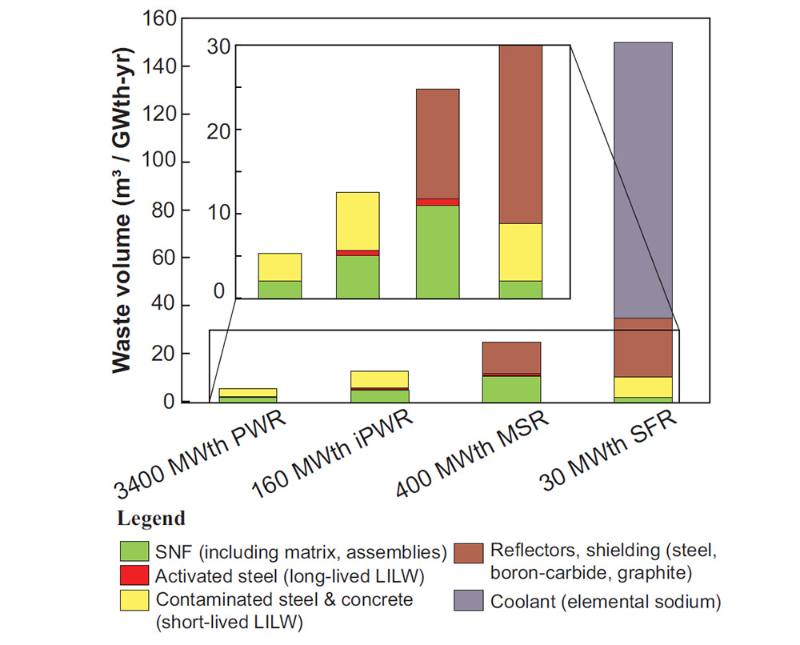
Problems associated with nuclear power reactors may occur throughout the plant’s operation, and SMRs can produce radioactive waste. Stanford University conducted research on whether or not the smaller design would result in less radioactive waste in 2022. The end consequence is that the inverse is correct. Even though each reactor generates less nuclear waste on its own, the total quantity generated is much higher.
Aside from using more fuel per unit of electricity, tiny power plants also generate a lot more radioactively contaminated structural substances. This is in comparison to a traditional pressurized water reactor. Graphite shields and reflectors join the ranks of steel and concrete buildings. Fast breeder reactors seem to be in the worst shape. Final storage requires the complete removal of all sodium used as a coolant.
The waste from small modular reactors (SMRs) is not chemically identical to that from a major nuclear power station, which is another issue. Instead, the contemporary castors have such a high concentration of radioactive elements (uranium-235 and plutonium) that a critical mass might be reached, setting off the reaction once again.